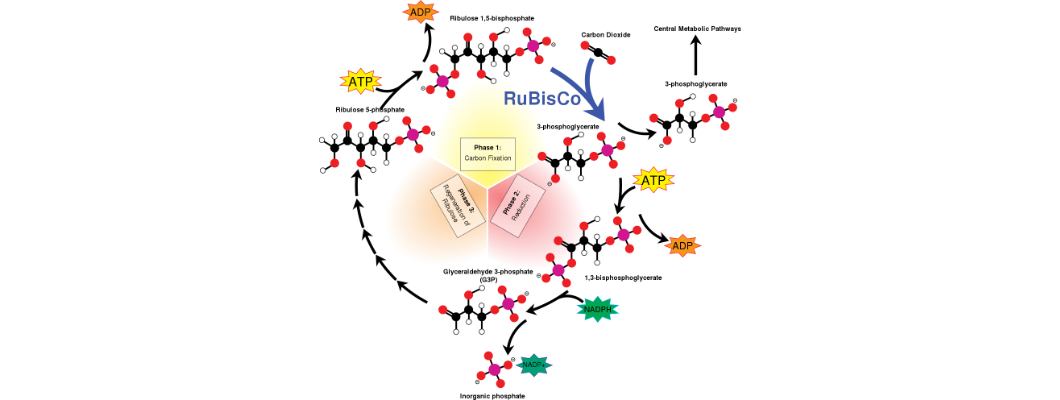
Title image above is attributed to Mike Jones, CC BY-SA 3.0 , via Wikimedia Commons
(This article was originally published 23rd February 2023 here on our Jujube Tree Nursery site.)
First published here 28th February 2023.
Please note that this post is somewhat technical, and assumes some of the writing done on photosynthesis here, and especially here.
Earth’s Atmospheric Carbon Dioxide (CO2) Concentrations Over Time
The previous post covered the fall of atmospheric carbon dioxide (CO2) from 97% [970,000 parts per million (ppm)] 4.5 billion years ago, to around 4% (4,000 ppm) 500 million years ago when plants appeared, to 0.2% (2,000 ppm) 60 million years ago, to 0.05% (500 ppm) 24 million years ago, to 0.04% (400 ppm) today.
It ended with the suggestion that plants — which have been around for 500 million years — may actually be starving in today’s world.
Let’s explore this concept further, in the context of evolution.
Carbon Fixation
Carbon fixation is the conversion of inorganic carbon (typically CO2) into organic carbon (carbohydrates). Only autotrophs can fix carbon, and are the sole means by which organic carbon becomes available to all other life (the heterotrophs). The first life-forms were probably microbial autotrophs.
Plants and algae are the only autotrophs in our macro (visible) world. All animals are heterotrophs, and can only obtain organic carbon via plants, or other animals, or both. (Plants do also undertake heterotrophy, so as to process the carbohydrates they made.)
(Because plants and algae also photosynthesise, it’s easy to assume autotrophy is synonymous with photosynthesis — and technically this is true for the macro world. But there are many autotrophic microbial species which fix carbon via other routes that have nothing to do with the sun. Those which fix carbon from CO2 emissions from deep underwater vents are the classic example here.)
The Calvin Cycle
90% of all carbon fixing is via the Calvin cycle. This is the light-independent part of photosynthesis, in that photons are not required as an energy input.
The enzyme ribulose-1,5-bisphosphate carboxylase-oxygenase (RuBisCO for short) — possibly the most common enzyme on the planet — initiates this cycle by catalysing the attachment of a CO2 molecule to another molecule called ribulose 1,5-bisphosphate (RuBP). It does this in conjunction with the ATP and NADPH collected via the light-dependent reactions of photosynthesis.
RuBP is the CO2 acceptor, and together with RuBisCO is the predominant means by which abiotic carbon enters the living world.
The attachment of one molecule of CO2 to the five-carbon RuBP makes a very unstable six-carbon molecule called 2-carboxy 3-keto 1,5-biphosphoribotol (CKABP), which immediately splits into two three-carbon molecules called 3-phosphoglycerate (PGA).
One of the PGA molecules stays in the Calvin cycle to ultimately regenerate more RuBP, while the other enters a completely different metabolic pathway to form sugars.
The whole process is summarised here:
The Calvin Cycle
Attribution: Attribution: Mike Jones, CC BY-SA 3.0 , via Wikimedia Commons
C3 Carbon Fixation, C4 Carbon Fixation and Crassulacean Acid Metabolism (CAM)
There are three known metabolic pathways of carbon fixation via photosynthesis: C3 carbon fixation, C4 carbon fixation, and Crassulacean acid metabolism (CAM). To know of these not only provides incredible insight as to the evolution of plants shaped by planetary changes over vast timespans, but also helps understand why different species perform differently in hot weather. Let’s begin!
C3 Carbon Fixation
C3 carbon fixation is simply the first step in the Calvin cycle as described above — the conversion of CO2 and RuBP into two molecules of PGA:
CO2 + H2O + RuBP → (2) 3-phosphoglycerate
This is by far the most common carbon-fixing pathway, found in about 90% of all plant species today. It is believed to have arisen from cyanobacterial endosymbiosis around 1-2 billion years ago.
C3 plants were the first plants to appear some 500 million years ago, and today thrive in areas of moderate light intensity, moderate temperatures, and plenty of water. They include important carbohydrate food crops such as rice, barley and wheat.
C3 plants prefer CO2 levels of 200 ppm or higher, and the reason they do not grow in very hot areas is related to a declining atmospheric concentration of CO2. When these plants first appeared some 500 million years ago, CO2 levels were about 4,000 ppm, or ten times higher than currently. However, exposure to higher temperatures in the presence of today’s lower CO2 concentrations of just 400 ppm causes the undesirable phenomenon of photorespiration.
Photorespiration is where RuBisCO adds oxygen to RuPB instead of CO2, and this rate of incorporation increases as temperature increases. This oxygenated RuBP cannot be used in the Calvin cycle, and results in wasted energy from photosynthesis, a reduced rate of PGA synthesis, and ultimately a net loss of carbon and nitrogen (as ammonia, NH3) to the plant. All this of course leads to reduced growth.
Further complications arise with the uptake of water by the roots, known as transpiration. Transpiration is a completely passive process driven by capillary action through the xylem, and 97% or more of uptaken water is lost through the leaves. Transpiration is a physical process rather than a physiological one.
C3 plants have no control over this entirely physical driver of water movement except to close their stomata (leaf pores) in hot weather so as to reduce this loss. However, this also means no CO2 can enter. A loss of CO2 inside the leaf further distorts the CO2:O2 ratio and leads to still more photorespiration.
C4 Carbon Fixation
C4 plants are relatively new in evolutionary history, possibly appearing around 25-32 million years ago. (CO2 levels were about 500 ppm or 0.05% by 24 million years ago.) They make up about 3% of all plant species.
C4 plants are able to concentrate CO2 around RuBisCO through a leaf modification called kranz anatomy. This prevents photorespiration and gives C4 plants a competitive advantage over C3 plants in both hotter and drier climates. Such plants are mostly found in the tropics and subtropics, all are flowering (the angiosperms), and most are grasses. Important C4 carbohydrate food crops include maize, sorghum, sugar cane, and some millets.
C4 fixation is in addition to C3 fixation. CO2 is first fixed into a four-carbon molecule (either malate or aspartate), which then moves to bundle sheath cells within the leaf. There, the malate or aspartate is broken down into CO2 and pyruvate. The CO2 enters the Calvin cycle and the pyruvate is reused to make more malate or aspartate.
C4 plants are better adapted to drought, high temperatures, and low CO2 levels.
Crassulacean Acid Metabolism (CAM)
Crassulacean acid metabolism (CAM) evolved as a water-conserving adaptation in arid conditions. Succulent lovers may even recognise the succulent family Crassulaceae in the name, in which this process was first discovered!
The majority of plants with CAM are epiphytes, which grow on trees but do not harm them (eg orchids and bromeliads), or xerophytes, which grow in very dry environments such as deserts (eg cacti and the Euphorbia genus). CAM is in about 7% of all species, and in over 99% of the Cactaceae (cactus) family of about 1,750 species.
Some aquatic species utilise CAM, not because of a lack of water, but for a lack of CO2, which has a 10,000 times lower diffusion rate in water than in air.
As with the C4 pathway, the CAM pathway is additional to the C3 pathway, also fixes malate, and also increases the concentration of CO2 around the RuBisCO enzyme to remove photorespiration and increase photosynthetic efficiency.
Where CAM differs however, is that plants with this metabolism completely close their stomata during the day so as to conserve maximum water. They open their stomata at night to allow CO2 to enter, which they then fix into malate during the night. Again different to C4 plants, this malate is then stored in leaf vacuoles ready for the Calvin cycle during the day. (The Calvin cycle, though it makes up the light-independent reactions of photosynthesis, cannot run at night. This is because it still needs the short-lived NADPH generated by the light-dependent reactions during the day.)
Are Plants Starving?
That the Calvin cycle and C3 pathway was the only method of carbon fixation in plants for some 470 million years — and acquired via a symbiosis with cyanobacteria over 500 million years prior — implies that this was a very successful mechanism. One could further argue that cyanobacteria, which first appeared about a billion years before that symbiosis, had had plenty of time in which to refine the Calvin cycle by then.
RuBisCO is the sole enzyme responsible for carbon fixation in plants (and cyanobacteria), by pulling CO2 molecules (inorganic carbon) out of the air and into the Calvin cycle, which enables carbohydrate manufacture elsewhere.
The ‘C’ in RuBisCO stands for ‘carboxylase’. A carboxylase is an enzyme which adds a CO2 molecule to another. RuBisCO carboxylates RuBP.
The ‘O’ in RuBisCO stands for ‘oxygenase’. An oxygenase is an enzyme which oxidises a molecule by transferring oxygen from oxygen gas (O2) to it. RuBisCO also oygenates (oxidises) RuBP.
In other words, RuBisCO is an enzyme which is both a carboxylase and an oxygenase — it’s in its very name.
Yet why RuBisCO acts as an oxygenase at all is actually quite puzzling, as C3 plants — by far the the oldest — are susceptible to photorespiration today because of that oxygenase activity.
RuBisCO, an enzyme which evolved to fix carbon, came into existence at a time of much higher CO2 levels. It is possible that its oxygenase activity had a role in the distant past which was advantageous then but which makes it a liability now. And, because of its corresponding carboxylase activity, that this involves CO2 concentrations somehow. Perhaps runaway carbohydrate production during peak photosynthesis in the presence of higher CO2 led to feedback mechanisms that switched on RuBisCO’s oxygenase activity? And that this is turn acted as a damper, or brake, on the Calvin cycle to slow everything down?
(There are many examples of negative feedback loops in organisms — regulation of body temperature is but one.)
If true, then it makes sense that an enzyme, which evolved millennia ago in (and probably for) high atmospheric CO2, could cause unintended consequences such as photorespiration in today’s new normal of low atmospheric CO2.
The C4 and CAM pathways are both quite elaborate processes, and all for the single purpose of increasing the concentration of CO2 around the RuBisCO enzyme. For C3 species worldwide to evolve not just one, but two mechanisms at all, implies significant environmental pressure on plants to justify these energy-intensive and complex systems.
Atmospheric CO2 was declining, from around 2,000 ppm (0.2%) 60 million years ago, to 500 ppm (0.05%) around 24 million years ago (possibly due to lower volcanic activity).
The C4 and CAM pathways were already appearing by 30 million years ago, but was this in response to rising temperatures, increasing aridity, lower CO2, or some combination of the three?
Dropping CO2 levels would have been global, but increasing aridity and/or temperatures would have been more localised and due to plate tectonics, continental drift, and the reduction of the shallow inland lakes and seas more common during the rise and proliferation of C3 plants. Species in regions becoming increasingly arid, tropical, or arid-tropical would have had much selective pressure placed on them over millennia to evolve a means of photosynthesising in ever increasing temperatures and decreasing CO2 and water. This would naturally lead to the rise of C4 and CAM species in these areas.
In contrast, C3 species in temperate zones would have had less selective pressure placed on them, as temperatures and water availability remained favourable for them. Even at high temperatures C3 plants could still flourish, providing CO2 levels were still higher, as RuBisco begins to favour oxygen over CO2 at high temperatures and lower CO2. And to this day the C3 group remains the vast majority of all plant species at around 90%.
Yet photorespiration is very much a negative factor for C3 species, which demonstrably do better at higher CO2 concentrations:
Effect of additional CO2 on Eldarica pines (P. brutia subsp. eldarica)
Attribution: unknown, but slide is viewable on the video ‘NASA Engineer Tom Moser Reveals the Truth About Climate Science’ at this timestamp: https://youtu.be/1-gHWcrCK7w?t=788
Does this imply that C3 plants are in a perpetual state of starvation, barely clinging to life, unable to adapt as their C4 and CAM cousins did so long ago? This is probably not quite what is going on. Just from casual observation you’d be hard-pressed to look around any temperate environment of lush green foliage and claim the plant life is forever sick, struggling, or on the verge of collapse.
And the sheer bulk of C3 biomass still here attests to that — 24 million years of low CO2 levels couldn’t wipe them out.
Perhaps it is not so much that the C3 plants are in a state of starvation, but rather are in a state of borderline malnourishment. (Just as many people have borderline unknown, undiagnosed nutrient deficiencies, yet are seemingly in good health.)
Anyone with a garden, orchard, or nothing but weeds knows how quickly everything takes off after a good run of warm sunny days and good rain. (An interesting thought: CO2 is heavier than air. Does the high humidity of rainy weather temporarily keep CO2 closer to the ground for plants to access?)
C3 plants may be thriving in bursts of great weather but getting by just well enough in not-so-great weather season-to-season, decade-to-decade, millenium-to-millenium, that pressure to evolve a parallel C4 or CAM pathway is not strong enough. Much like someone knowing they’re marginally deficient in something but not motivated enough to do anything about it.
Atmospheric CO2 was around 275 ppm (0.0275%) in the early 1600s, about 34% lower than today. NASA data shows the Earth greening significantly as CO2 levels have risen. Mini fluctuations of CO2 like this through time may further lessen any urgency to adapt.
(In truth, there are additional factors at play here which involve solar cycles — see here and here if interested.)
We may never know how many C3 species did become extinct as CO2 levels dropped. And no doubt new species arose during lower CO2 concentrations, which never knew higher levels. But what of species today whose ancestors can be traced back to the first appearance of plants 500 million years ago?
Mosses are the oldest plant species in the world, dating back to 470 million years ago. The Agathis genus, which includes the New Zealand kauri (one of the world’s largest trees) and other Australian and Malesian kauris, is the second-oldest and dates to 300-360 million years ago. These represent amongst the smallest and the largest of all C3 species, and it would be fascinating to observe how these species would respond to the higher concentrations their ancestors experienced.
Leave a Comment